Most commercial solar cells achieve 15-25% efficiencies, converting incoming solar energy into usable electricity. But a new record has been published in 2020, achieving 47.1% conversion efficiency. The paper used “a monolithic, series-connected, six-junction inverted metamorphic structure under 143 suns concentration”. Our goal in this short note is to explain this solar efficiency record.
“A monolithic, series-connected, six-junction inverted metamorphic structure under 143 suns concentration”
p-n junctions are the foundation of all solar cells. Each side of the junction is doped, so that the โnโ-side will surrender electrons, while the โpโ-side will accept electrons. When incoming solar energy strikes the junction, it may dislodge an electron and leave behind a hole. The liberated electron will propagate towards the โpโ-side, while the holes will propagate towards the โnโ-side, thus creating a direct current.
Bandgap is the energy needed to dislodge an electron from its usual orbit, so it is free to move through a p-n junction. The energy in light varies with wavelength (lower-wavelength equals lower energy). Light waves below the bandgap will not suffice to dislodge electrons: they will pass through the material and the energy will not be captured. Light waves above the bandgap will have excess energy left over after dislodging electrons: the excess energy will be lost as heat.
Single-junction solar cells are composed of p-n junctions made of a single material, most commonly crystalline silicon, in today’s commercial solar industry. Silicon atoms have a bandgap of 1.4eV and achieve optimum conversion efficiency in light with 700-1,000nm wavelengths (red and infra-red). They do not capture energy efficiently from lower energy, lower wavelength light (such as 400-700nm) or very high wavelength light (1,000nm+).
Multi-Junction solar cells aim to overcome the limitations of single-junction solar cells, combining multiple p-n junctions, made of multiple solar materials, to capture a broader range of the spectrum. For example, the six-junction solar cell discussed in this note has six separate junctions, connected in series, to capture light from c350-1700nm wavelengths, which is tantamount to c65-85% of all the energy in sunlight.
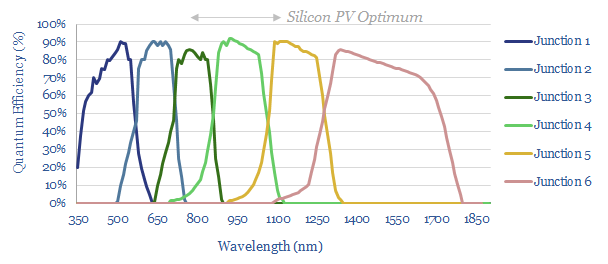
Group III-V alloys are used in different combinations in each of these junctions, to tune its bandgap, to capture a different wavelength of light. These alloys are composed of elements from Groups III and V in the periodic table. Group III includes boron, aluminium, gallium and indium. Group V includes nitrogen, phosphorus, arsenic and antimony.
The junctions are usually stacked with the highest energy absorber on top (i.e., junction 6). Photons that lack sufficient energy to dislodged electrons in junction 6 will pass through it, and have a additional chances of being absorbed in junction 5, through to junction 1.
The challenge is how to stack these six junctions on top of each other in a way that limits recombination and resistance, both of which are going to impair solar cell efficiency.
The challenge of recombination?
Recombination occurs when dislodged electron and holes re-combine in a solar cell, thereby lowering the current reaching the current collectors. If recombination re-emits photons, it is known as radiative recombination. Group III-V solar cells are particularly sensitive to recombination around dislocations.
Dislocations are abrupt changes in the crystal structures in a material. A physical effect is that dislocations allow atoms to glide or slip past one another at low stress levels. An optoelectronic effect is to impede current and encourage recombination of electrons and holes.
One type of dislocation, known as a threading dislocation because of its shape, extends beyond the surface of the strained layer and throughout the material, so it can be particularly deleterious to solar cell performance.
Multi-junction solar cells are particularly prone to dislocations because each junction is made of a different material. These materials are lattice-mismatched monoliths.
Monolithic materials are formed a single, continuous and unbroken crystal structure, all the way to its edges, with minimal defects or grain boundaries. This means it does not suffer from grain boundaries or dislocations, and in turn, efficiency losses from recombination should be minimized. But it is very difficult to manufacture monolithic materials from lattice-mismatched components.
Lattice-mismatched materials have different lattice constants. This means that they are composed of crystals of different sizes. In turn, this means they will not adhere well to one-another. Their boundaries are prone dislocations.
The solution: metamorphic epitaxy?
A technique called metamorphic epitaxy was used to create the monolithic six-junction solar cells described above, and overcome the inter-related challenges of recobination at dislocations in lattice mismatched materials.
Epitaxy is the process of orientation-controlled growth of crystals on top of other crystals. The 47% efficient solar cell used a variant called organometallic vapour phase epitaxy (OMVPE). Our overview of manufacturing methods is here.
Metamorphic epitaxy minimizes dislocations around the active site of an engineered material. This is achieved by relieving the strain around lattice-mismatched boundaries by encouraging dislocations to occur away from the active site of the material. Specifically, materials known as Compositionally Graded Buffers (CGBs) were introduced in between the fourth to sixth junctions of the six-junction solar cell, as thse were the boundaries most prone to dislocations.
Specifically, these six-junction solar cells were monolithically grown on a single 2×3 cm GaAs substrate, at 550-750C temperatures, in an atmospheric-pressure OMVPE system. โGrowth begins with the high-bandgap lattice-matched junctions [on the bottom], leaving these high-power-producing junctions without dislocationsโ.
Then the cell was then inverted as the high bandgap lattices need to be situated on the top of the cell. (In other words, the cell is printed upside down and then turned over). Gold was electroplated onto contact of the inverted structure (literally, “gold-plated”!), then the cell was epoxied onto a flat silicon wafer. The GaAs substrate was removed by chemical etching. A front-side grid of NiAu was deposited by photolithography. Finally an anti-reflective coating of MgF2/ZnS/MgF2/ZnS was thermally deposited on the top of the cell.
The full 6J IMM structure consisted of 140 layers, including individual compositional step-graded buffer layers. The total growth time was 7.5โh.
1 sun’s concentration?
Under 1 sun’s solar intensity, the cell described above achieved 39.2% efficiency. This is the highest 1-Sun conversion efficiency demonstrated by any technology to-date. The prior record is 38.8% for a five-junction bonded IIIโV solar cell.
The efficiency is very high, because the voltages of each junction add up to a high total voltage. However, the current density in each junction was low. The efficiency could have been higher with a higher current density, which in turn, is achieved by concentrating the incoming sunlight.
143 suns’ concentration?
Concentration of incident light improves solar cell efficiency. The reason is that more concentrated light dislodges more electrons. More dislodged electrons means a higher current density. In turn, a higher current density raises the bandgap for dislodging further electrons (it is harder to remove further electrons from a material that has already lost some electrons). So even more energy can be absorbed when additional light strikes the cell.
Concentrating solar light is also desirable as a way to lower costs, as multi-junction solar cells are expensive to produce. Concentrating the light from 1 square meter onto 1 square centimeter, for example, reduces the area of solar materials required by a factor of 10,000.
Joule losses set the upper limit on the solar concentration that will maximize efficiency. Joule losses are the loss of electricity as heat when electric current passes through a conductor. They are a square function of current and a linear function of resistance. So they rise quadratically as solar intensity rises linearly.
Lower resistance will help to limit joule losses. In the solar cell described above, several challenges were observed keeping resistance low.
Each junction is connected in series in the cell. The current flows between each junction through a “tunnel interconnection”. Resistance through these tunnel junctions was found to rise with current, placing a practical limit on solar concentrations.
Internal resistances within each junction were also higher than desired. They were found to have been elevated by the temperatures during epitaxy and during dopant diffusion (particularly in Zn-containing layers).
At the top junction, the 2.1eV bandgap material required a high resistance to conduct charge laterally to the metal grid fingers that serve as current collectors for the cell’s electrical circuit.
Reducing the effective series resistance to 0.015โฮฉโcm2 is seen to be possible, by analogy to previous four-junction solar cells, which would allow the six-junction cell described above to surpass 50% efficiency at 1,000โ2,000 Suns. The maximum theoretical efficiency is 62%.
Commercial implications?
47-50% efficient solar cells are a good incremental improvement. To put the ‘breakthrough’ into context, the previous record for a multi-junction solar cell was 46% efficiency at 508 suns, using a four-junction device. There is scope for multi-junction solar cell efficiency to improve further.
The cell was also very small, at 0.1cm2. When solar ‘records’ are measured, usually the stipulation is required that a cell must be 1cm2 in area, as a testing criterion.
Its production was very complex taking 7.5-hours to assemble 140 separate layers. Complex structures are expensive and more prone to degradation, which makes commerciality challenging.
We conclude that a 47-50% efficient solar cell is a tremendous technical achievement. But the evidence does not yet suggest proximity to commercialising ultra-efficient multi-junction solar cells like this at mass scale. All of our solar research is summarized here.
Source: Geisz, J. F., France, R. M., Schulte, K. L., Steiner, M. A., Norman, A. G., Guthrey, H. L., Young, M. R., Song, T. & Moriarty, T. (2020). Six-junction IIIโV solar cells with 47.1% conversion efficiency under 143 Suns concentration. National Renewable Energy Laboratory (NREL), Golden, CO, USA.